|
FIG PUBLICATION NO. 49
Cost Effective GNSS Positioning Techniques
FIG Commission 5 Publication
Dr. Neil D. Weston, United States
Dr. Volker Schwieger, Germany
Contents
Foreword
1. Introduction
2. Global Navigation Satellite Systems
2.1 Introduction
2.2 GPS
2.2.1 Introduction
2.2.2 GPS Signal Structure
2.2.3 GPS System Time
2.3 GLONASS
2.3.1 Introduction
2.3.2 Signal Structure
2.3.3 GLONASS in the Future
2.4 GALILEO
2.4.1 Introduction
2.4.2 Galileo Signals
2.4.3 Galileo Services
2.5 COMPASS
3. GNSS Positioning Techniques for Surveying
3.1 Introduction
3.2 Relative Positioning
3.3 Precise Point Positioning
3.4 Positioning Software Packages and Data Types
4. Cost-effective GNSS
4.1 Introduction
4.2 Cost-Effective Rovers / Low-Cost GNSS Receivers
4.3 Continuously Operating Reference Station (CORS) Networks
4.3.1 Introduction
4.3.2 CORS Station Configuration
4.3.3 Products from a CORS Network
4.4 Web-based Positioning Tools
4.4.1 Online Positioning User Service
– OPUS
4.4.2 Scripps Coordinate Update Tool –
SCOUT
4.4.3 AUSLIG’s Online GPS Processing
Service – AUSPOS
4.4.4 Canadian Spatial Reference System
Online Global GPS Processing Service – CSRS-PPP
5. Cost-effectiveness
6. Concluding Remarks
Appendix A - The International GNSS
Service
Appendix B - Additional Information and Resources
Appendix C - Global and Regional Reference Station
Networks
Bibliography
Orders for printed copies
Most surveyors today are aware that data acquisition, management,
analysis, presentation and controlling systems are becoming more elaborate
and automated. The International Federation of Surveyors (FIG) Commission 5
– Positioning and Measurement, has been and remains an essential part of the
surveying community’s growth and development. During the past decade, Global
Navigation Satellite Systems (GNSS) have and continue to play an
increasingly important role in positioning and navigation.
The FIG enhances this development by facilitating GNSS sessions at their
conferences, encouraging GNSS research and education, and cooperating with
sister organisations such as the International Association of Geodesy (IAG) in
the respective domain. Additionally, the FIG is also trying to integrate GNSS
surveying as a base and starting point for land administration as well as
cadastral registration, especially in developing countries regarding property
evidence. This is one of the major reasons why FIG is looking at cost-effective
technologies and techniques for enabling surveyors in developing countries to
use the best equipment at a reasonable price. This Technical Report focuses on
the cost-effective use of GNSS. It originates with the help of Special Study
Group “Cost-Effective GNSS” within the Working Group 5.4 “GNSS”.
As Chair of FIG Commission 5 – Positioning and Measurement, I thank Dr.
Neil Weston, National Geodetic Survey, USA and Dr. Volker Schwieger,
University of Stuttgart, Germany for their efforts in compiling this report.
Prof. Dr.-Ing. Rudolf Staiger
Chair FIG Commission 5
January 2010 |
|
1. Introduction
Global Navigation Satellite Systems (GNSS) were initially
developed in the early seventies to improve global positioning and navigation
from space. The Global Positioning System (GPS) was the first system to launch
an operational prototype satellite in February of 1978. Shortly after, the
number of GPS satellites in orbit increased to four but this was the absolute
minimum to obtain a “fix”. More satellites would be needed if continuous global
coverage was expected. GNSS constellations are constantly being expanded and
upgraded but many of the initial designs and integrated systems on the original
satellite are still found on newer satellites in the current GPS constellation.
The first commercial GPS receivers were on the market in 1982.
The receivers were large and bulky and could only track four satellites
simultaneously. The satellites to track had to be selected manually on the
receiver. Moreover, national geodetic agencies, research institutions and
universities spent up to 250,000 € for a single receiver. Today, modern
receivers are much more sophisticated and can track GPS and GLONASS satellites
simultaneously on more than 50 channels. Some of the latest receiver models can
also track Galileo signals. Everything from satellite tracking to coordinate
determination are computed automatically in real time. At the same time costs of
new receivers continue to decrease. A high-end geodetic quality GNSS receiver
costs around 20,000 €. If a user is restricted to single-frequency, geodetic
quality receivers, one would still have to spend 5,000 € to 12,000 €. In general
this does not pose a problem in developed countries, but it may be a drawback in
developing countries or for tasks where the surveyor needs a lot of receivers
for specialized tasks such as monitoring.
In this FIG report the authors will present several topics on the
cost-effective use of GNSS. There are two possibilities to economize resources.
The first pertains to a reference site or a network of reference stations and
the second primarily concentrates on the rover or users side. For the first, we
initially focus on Continuously Operating References Station (CORS) networks
that provide the reference site(s) data and metadata to the users. For the
second, the report proposes to use low-cost (below 150 €) GNSS receivers instead
of high-end geodetic quality receivers. After giving an overview on GNSS and
geodetic positioning, both approaches and their opportunities are presented.
Finally, several cases on estimating the working costs will be developed and
analyzed.
2. Global Navigation Satellite Systems
2.1 Introduction
In the modern age of positioning and navigation, satellites from
any of the Global Navigation Satellite Systems (GNSS) can be used to accurately
position stationary or moving objects. This general approach is often termed
satellite positioning. The basic idea is to determine the position of an antenna
(Ri(t)) connected to a GNSS receiver where the antenna could be over a
survey marker, on a plane or ship, or even be a part of a GNSS reference
network. Since the positions of all the satellites in a constellation can be
calculated at any time t and the range between any visible satellite and
the antenna is measured by a GNSS receiver
(ρj
i(t)),
the only remaining unknown is the position vector from the origin of a common
coordinate system to the antenna which is being positioned. Fig. 1 shows the
basic concept of satellite positioning using a single satellite j and an
Earth Centered Earth Fixed (ECEF) reference frame.
In practice however, accurate positioning of objects with GNSS
satellites is much more complex. If we look at a constellation of satellites
first, a user needs to know the exact position of each of the space vehicles (Rj(t))
during a period of time or in the near future. Satellite positions as a function
of time, otherwise known as ephemerides, were initially determined by groups
working in spacecraft dynamics, aeronautics and celestial mechanics. Today there
are quite a few research and academic institutions which are actively involved
in computing satellite ephemerides. The International GNSS Service (IGS), for
example, uses a global network of reference stations to track the position and
estimate the orbit of each satellite using a weighted least squares process
(Beutler et al. 1999, 2005, 2009, Dow et al. 2009, Appendix B.1–2). Over the
last decade, the orbit determination process at the IGS Analysis Centers has
improved significantly and accuracies better than a few centimeters for the IGS
Rapid and Final orbit products are now routinely achieved. Appendix A lists the
orbit products and their accuracies which are currently available through the
IGS.
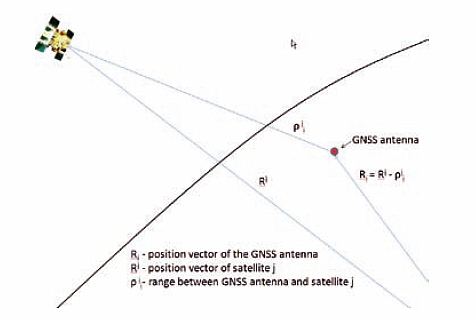
Fig. 1: Satellite positioning using an ECEF reference frame.
Another major area for discussion has to do with how the range (ρj
i(t)) between satellite j and receiver i is measured. Different
positioning techniques have evolved based on various operating conditions, but
all use the following basic equation to estimate the position vector Ri(t)
for an antenna.
|| Rj(t) – Ri(t)
|| = ρj i(t) i,j = 1,2,3,...
(2.1)
For stationary objects, the most common positioning techniques
are precise point positioning (PPP) and relative or differential positioning.
For these cases, a user can compute a position every epoch and under ideal
conditions, should get redundant results. As a second method, a user could also
compute a single set of coordinates based on many epochs, either through
averaging or with a least squares approach (Strang and Borre 1997, Leick 2004).
If an antenna is on a moving platform, then a position has to be
computed at every epoch and is often referred to as kinematic positioning. Two
frequently used techniques under this category are point positioning and
real-time kinematic positioning, in either a point positioning mode or relative
to one or more stationary receivers. These approaches are often augmented by
additional ground or space-based systems such as the wide area augmentation
system (WAAS) used primarily by the aviation industry and the differential GPS
(DGPS) system initially implemented by the United States Coast Guard.
Many augmentation systems such as the WAAS system use
geostationary satellites to broadcast differential correctors to receivers for
improving the positioning capabilities as well as enhancing the integrity of the
overall system. The Coast Guard’s DGPS system was initially designed to support
the maritime communities along the coasts and inland waterways of the United
States, but because of its performance and popularity, the system is now being
expanded across the nation and is being referred to as the Nationwide
Differential Global Positioning System (NDGPS). Similar augmentation systems
have also been developed and are currently operational in Europe and Japan. The
European Geostationary Navigation Overlay Service (EGNOS) uses three
geostationary satellites and numerous ground stations to enhance critical
navigation applications used by civilian and military aircraft and ships in
Europe. The MTSAT augmentation system being developed by Japan will support the
country’s meteorological agency as well as the aviation sectors and will provide
coverage over East Asia and the Western Pacific.
2.2.1 Introduction
The Navigation Satellite Timing and Ranging (NAVSTAR) Global
Positioning System (GPS) was developed by the United States Department of
Defense (DoD) to provide worldwide positioning and timing capabilities for the
military. The system was initially designed to provide two types of services.
The first is the precise positioning service (PPS) and is for military use while
the second is the standard positioning service (SPS) and is intended for use by
everyone (SPS, 2001)
Different components of the GPS system fall into three main
groups. The first is known as the SPACE segment and consists of 24 operational
satellites built by Rockwell International, Lockheed Martin and Boeing. Four
atomic clocks on each satellite continuously compute and transmit the exact time
(GPS time) and position of the satellite in a digital signal. The satellites are
also placed, in equal numbers, in six orbital planes, each inclined at 55° from
the Earth’s equator. Each satellite moves in a near circular orbit (semi-major
axis 26,660 km) and completes two orbital revolutions in one sidereal day. The
inclination of the orbits and the high altitude (~20,000 km) of the GPS
satellites permit more to be seen simultaneously from virtually anywhere on
Earth. Satellite visibility is also optimized by monitoring and routinely
adjusting the positions of all satellites in each orbital plane.
The second major component of the GPS system is the CONTROL
segment. This currently consists of eleven monitoring stations where each
station monitors and accumulates the range to each visible satellite before
passing the information on to the Master Control Station (MCS) at Schriever AFB
in Colorado. The MCS is responsible for computing the orbit of each satellite
and to update the navigation or broadcast message with parameters that describe
each satellite’s orbit. The broadcast message is then sent to each satellite via
an up-link from one of three ground antennas located in Ascension Island, Diego
Garcia and Kwajalein.
The third component of the GPS system is the USER segment. This
primarily consists of GPS antennas and receivers that provide position,
navigation and timing information to the users. The number of applications for
GPS continues to rise and over the last decade, there has been a dramatic
increase in the number and type of receivers which have been developed and sold
for civilian and military uses.
2.2.2 GPS Signal Structure
One of the principle design features of all GPS satellites is to
use onboard atomic clocks to generate signal transmissions from the fundamental
frequency of 10.23 MHz. The two initial signals on all GPS satellites are known
as the L1 and L2 carriers and are multiples of the fundamental frequency. The L1
carrier is 154 times the fundamental frequency, f1 = 1575.42 MHz while the L2
carrier is 120 times the fundamental frequency, f2 = 1227.60 MHz. The encrypted
Precision or P(Y) codes on the L1 and L2 carriers have the same fundamental
frequency of 10.23 MHz, while the Coarse/Acquisition or C/A code has a chipping
rate of one-tenth the fundamental frequency, i.e., 1.023 MHz.
As part of the GPS modernisation effort, a second civil frequency
was added on L2 for Block IIR-M and later satellites launched after 1998. The
new navigation signal is referred to as L2C and aims to improve accuracy of
navigation with enhanced signal tracking capabilities. In March 2009, a Block
IIR-20(M) satellite was launched with the new L5 safety of life civil signal
(1176.45 MHz) and is the first in a series designed with higher transmitting
power, wider bandwidth and enhanced performance. The modernisation of the GPS
constellation aims to provide more transmitting signals to both the civilian and
military communities and is scheduled to continue through 2013.
2.2.3 GPS System Time
GPS system time is the time given by the composite clock which
includes monitoring stations and the satellite frequency standard. A master
clock for GPS time is constantly checked against a clock at the United States
Naval Observatory (USNO) and steered to UTC so the difference is no greater than
one microsecond. The navigation message which contains parameters that describe
the satellite orbits also has two parameters that specify the time offset and
the rate of drift between GPS time and UTC (USNO). UTC (USNO) is also
synchronized to be in agreement with the international benchmark
for UTC.
2.3 GLONASS
2.3.1 Introduction
The Global Navigation Satellite System (GLONASS – GLObal’naya
Navigatsionnaya Sputnikovaya Sistema) is managed by the
Russian Space Forces for the Russian Federation Government. All operational
components of the GLONASS system are operated by the Coordination Scientific
Information Center (KNITs) which is a part of the Ministry of Defense of the
Russian Federation. Initial GLONASS development began in 1976 in the former
Soviet Union and was designed to be an alternative to the GPS system offered by
the United States. There have been several generations of satellites in the
GLONASS constellation. The two most recent, which are known as GLONASS-M and
GLONASSK, have an estimated operational life span of 7 and 12 years
respectively. All satellites have atomic clocks and provide real time position
and velocity determination once a receiver has locked on and remains in signal
tracking mode. During the early operational stages, the horizontal positional
accuracy varied between 50–70 meters while the vertical accuracy was closer to
70 meters.
The space segment of GLONASS consists of 24 satellite slots in
three orbital planes separated by 120° and inclined at 64.8° with respect to the
equator. The eight satellite slots in each plane, numbered 1–8 for plane one,
9–16 for plane two etc., have a separation of 45°, a near circular orbit with a
period of 11 hours and 15 minutes, and have an altitude of 19,100 km above the
Earth. The spatial arrangement of the satellites in the three planes is such
that only one crosses the equator at a time and therefore a minimum of five can
be seen at any time, from any location on Earth. Any specific GLONASS satellite
will therefore pass over the same spot on Earth every eight sidereal days while
each GPS satellite passes over the same spot once every sidereal day.
The GLONASS control segment has two primary divisions. The first
is the Ground Control Center located in Moscow and the second are the telemetry
and tracking stations located at St. Petersburg, Eniseisk, Ternopol and
Komsomolsk-na-Amure. The GLONASS operating authorities also have active
expansion plans which include additional monitoring and tracking stations in
Australia, Cuba and South America to enhance the accuracy, reliability and
integrity of the system.
As of January 2010, the GLONASS system consists of 19 operational
satellites with two additional satellites listed as „in maintenance“. The
Russian territory has complete coverage with 19 satellites but for 100% global
coverage with five or more satellites in view, 24 satellites need to be
operational.
2.3.2 Signal Structure
There are two types of signals which are transmitted from the
GLONASS satellites. The first is the standard precision (SP) signal which is
transmitted between 316–500 Watts in a 38° cone using right-hand circular
polarization. Each satellite transmits the SP signal on the same code but uses a
different frequency. The L1 band is used with a technique known as frequency
division multiple access (FDMA) to assign different frequencies centered around
1602.0 MHz to 15 channels. The frequencies for the 15 channels are calculated by
using the following formula 1602.0 MHz + 0.5625 MHz x n where n is an integer
value from –7 to 7. The frequency for channel 0 would therefore be 1602.0 MHz
while the frequency for channel –7 would be 1595.56 MHz. The horizontal
accuracy, using the SP signal from four older GLONASS (first generation)
satellites, was typically between 5–10 meters while the vertical accuracy was
about 15 meters.
The second signal known as the high precision (HP) signal shares
the same carrier wave as the SP signal but uses a bandwidth which is 10 times
larger. The HP signal is primarily used by the Russian military and other
sectors with authorized access. The FDMA technique is also used to assign 15 L2
frequencies of the HP signal but now they are centered near 1246 MHz. In this
case, the same integer values used for n to calculate frequencies for L1 are
used for L2 but with the following formula 1246 MHz + 0.4375 MHz x n. Even
though the HP signal was broadcasted in a clear, un-modulated format in the
past, caution in using the signal is still suggested because a recently adopted
approach to broadcast 400bps at random intervals has been implemented on a
permanent basis.
2.3.3 GLONASS in the Future
A fairly significant change to parts of the GLONASS signal
structure are scheduled to take place when GLONASS-K (third generation)
satellites are added to the current constellation. These satellites will use
code division multiple access (CDMA) for L1 and L5 signals, a technology which
employs a coding scheme where each transmitter is assigned a unique code so
numerous users can be multiplexed over the same physical channel. The CDMA
approach will also begin to make the GLONASS constellation more compatible with
GPS and the future Galileo system.
2.4 GALILEO
2.4.1 Introduction
Galileo is formally known as the European Civil Satellite
Navigation Program and its start can be traced back to March, 2002 when the
European Council voted to declare the program as an official undertaking. The
Galileo Program has received most of its initial funding from numerous public
and private European institutions and is currently being developed as an
inter-operable counterpart to the GPS and GLONASS systems offered by the United
States and Russia.
The Galileo system is being designed with several major
operational segments. The first or global segment will contain 30 Medium Earth
Orbit (MEO) satellites, 27 operational and three spare, in three orbital planes
inclined at 56˚. In-plane satellites will be positioned at 40˚ intervals, have
an altitude of 23,222 km and will be maneuvered via velocity changes so orbit
period fluctuations are kept to an absolute minimum. The orbits were also chosen
to minimize gravitational resonances and to provide high visibility of the
satellites. Each satellite will transmit up to 10 navigation timing and data
signals, some of which will contain clock and ephemeris information to enable
worldwide positioning, navigation, timing and integrity monitoring services.
The ground control segment will be made up of five up-link
stations located around the world and will be responsible for the telemetry,
tracking and command (TTC) tasks for communicating with the satellites on a
regular basis. Two additional control centers located in Oberpfaffenhofen,
Germany and Rome, Italy will be responsible for analyzing and initiating
spacecraft control functions via the five TTC stations. Orbit maintenance and
systems monitoring activities will also be performed at the two European control
centers. A larger global network of up to 30 tracking stations will be used to
continuously monitor all satellite navigation signals in a redundant fashion.
Another significant component of the Galileo infrastructure is
the regional segment which will consist of numerous agencies within and outside
Europe that will offer integrity services independent of the Galileo system. The
integrity services, known as External Region Integrity Systems (ERIS), will also
be a part of a checking system used to legally monitor products, services and
guarantees offered by Galileo.
2.4.2 Galileo Signals
Each satellite in the Galileo constellation will use CDMA
technology to transmit up to 10 right hand circular polarized signals in the
frequency ranges 1164–1592 MHz. A specific code or key is added to each signal
so receivers can identify which satellite the signals are coming from and how
long the transmissions took. The more complex the code, the more time a receiver
spends in identifying which channel to assign for the signal. The satellite
identification codes also come in two formats. A long format code is more
difficult to acquire but improves tracking capabilities when signals are very
weak while short codes allow for very fast acquisitions.

Fig. 2: Galileo Signal Structure. (Courtesy Institute of Geodesy and
Navigation, University FAF Munich)
The range of the E5a band is 1164–1189 MHz and has 1176.45 MHz as
its central frequency. The same range is also used for the GPS L5 signal. The
E5b band is 1189–1214 MHz and uses 1207.14 MHz as the main frequency. This band
is equivalent to the GLONASS L3 band. The data on signals from the E5a and E5b
bands are partly encrypted and is available to all users.
The multi-lobed E6 band from 1260–1300 MHz is unique to Galileo.
It uses 1278.75 MHz as the central frequency to transmit signals which have
controlled access to the encrypted range and data information. Signals
associated with the side lobes of the E6 band are also encrypted and have
restricted access to the range and data information.
The final block of frequencies contain the E2, L1 and E1 signals.
This band ranges from 1559–1592 MHz, has a central frequency of 1575.42 MHz and
is used by both GPS and Galileo for the L1 signal. Figure 2 summarizes the
frequency spectrum, signal structure and data rates used by GPS and Galileo.
2.4.3 Galileo Services
One of the main reasons Galileo will offer up to 10 signals is to
try to meet the demands and requests brought forward by many current and future
GNSS users. Improving signal acquisition, tracking signals indoors, providing
codes with different signal characteristics and trying to improve the techniques
used to estimate the ionospheric delay are specific cases that would benefit
from having more signals. The signal structures and frequency allocations were
chosen by design so signals could be used in pairs, such as in determining the
ionospheric delay, where measurements using two different signals from the same
satellite can be determined and cancelled out. This effect is even more
pronounced as the separation between the frequencies of the two signals
increases.
With respect to common services such as positioning and
navigation, GPS and Galileo will have the same L1 and L5 signals and therefore
any increase in the number of satellites in space will strengthen the geometry
used to obtain a position.
In addition to the common services, there will be four or five
Galileo satellite-only services offered worldwide. The Open Service (OS)
provides position and timing capabilities, free of charge, to the worldwide
community. The performance of this service is on par with similar services
offered by other satellite constellations. The Public Regulated Service (PRS)
uses two signals and is offered to specific users who use high performance
positioning and timing applications that demand long continuity of service. The
Commercial Service (CS) offers similar features to select users but the signals
used in this case offer higher throughput rates and will be tailored for high
accuracy applications. The Safety of Life Service is designed to improve the
Open Service by providing integrity messages when performance falls below a
specified threshold. The last signal to be discussed in this section is the
Search and Rescue Service. Each Galileo satellite will be able to detect a
distress signal and pass on its location to a monitoring center in near-real
time thereby enabling rescue services more quickly. An acknowledgement or
feedback, in some cases, could be sent to two-way emergency beacons.
2.5 COMPASS
The United States, Russia and the European Community are not the
only countries to enter the global navigation and positioning race. China is
also developing an independent system to operate on a worldwide basis. Their
initial system is known as Beidou-1 and consists of four geostationary
satellites positioned primarily over Asia. Two satellites were launched in late
2000, a third in 2003 and the fourth in 2007. The experimental constellation
provides limited coverage that ranges from 70° E to 140° E and from 5° N to 55°
N. However two of the satellites are not usable and the status of a third is
unclear.
China’s new system known as Compass or Beidou-2 will have a
constellation of 35 satellites, will provide worldwide positioning and
navigation capabilities and will offer two levels of service. Five satellites
will be geostationary so the system is backward compatible with Beidou-1 while
the remaining satellites will reside in medium Earth orbits. The transmitting
signals will be based on code division multiple access (CDMA) technology and
will use frequencies from the E1, E2, E5B and E6 bands.
As of early 2009 two Compass satellites have been launched.
Compass-M1 was placed in orbit for testing of signals from the E2, E5B and E6
bands and to validate a number of service systems. A third Compass satellite was
later launched on January 17th of 2010. Implementation of a regional version of
the Compass GNSS system with 12 satellites is currently underway and is
scheduled to be complete by 2012. Funding to complete and operate the 35
satellite constellation by 2020 has been assured.
3. GNSS Positioning Techniques for Surveying
3.1 Introduction
Positioning of benchmarks and other stationary objects is
routinely referred to as static positioning while the positioning of moving
platforms such as a plane or a ship is referred to as kinematic positioning.
Since this report mainly addresses surveying, the authors will primarily focus
on static applications. Nevertheless kinematic problems may be solved in a
similar way. One very important point to note is that surveying applications
need accuracies that range between a few centimeters and a few millimeters. This
implies that phase observations have to be evaluated and each of the ambiguities
have to be solved. In this paper we cannot discuss all aspects of GNSS
positioning, however numerous textbooks are available to address advanced
topics. In the following sections, information regarding relative positioning
and precise point positioning are addressed since background information on
these topics are needed to understand the cost-effective techniques in chapter
four. Two additional topics will be addressed at this time. The first is that
the measurement quantities are referred to as pseudo-ranges since the ranges are
affected by the receiver clock error resulting in a pseudo-range. The
pseudo-ranges may be determined using code or carrier phase data. The term
pseudorange however, does not provide any decision with respect to how the code
or phase measurements were used, it is simply a general term. The second point
to mention is that a minimum of four pseudo-ranges are needed to determine the
position of an antenna, three for the coordinates and one for the receiver clock
error. This implies that a minimum of four satellites have to be tracked
simultaneously at each receiver-antenna combination to obtain a position at each
epoch.
3.2 Relative Positioning
The absolute accuracy of GNSS is about three to four meters if
the broadcast navigation message is used for positioning. In this case the
positions are determined with respect to the predicted satellite orbits. The
satellite broadcast elements are transferred to the user in real time via the
broadcast message which are modulated and transmitted on the GNSS signals. This
simplest positioning technique is known as absolute GNSS. This technique will
never reach the accuracy required for surveying tasks. There are two approaches
to obtaining highly accurate surveying results. The first is to use the carrier
phase data instead of the code data and the second is the use of differences
between measured pseudo-distances. The second technique is known as relative or
differential GNSS (DGNSS). If phase data is used we generally refer to this
technique as precise DGNSS. Common abbreviations are PDGNSS and PDGPS, but it
depends on which constellations are being used for the technique. The main error
sources of GNSS have to be identified to understand the advantages of
differencing. The principle error sources are associated with satellite orbits,
satellite clocks, ionosphere, troposphere, multipath, receiver clock and the
antenna phase centre. Figure 3 shows these main sources.
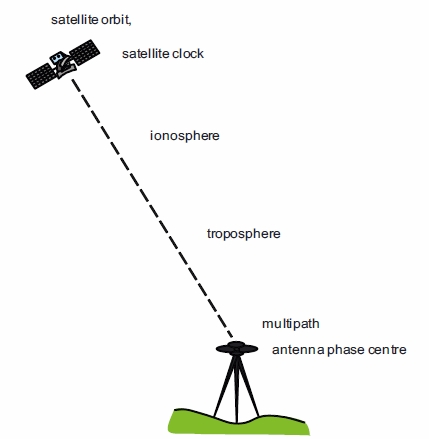
Fig. 3: GNSS main error sources.
The theory behind differential GNSS is the assumption that the
errors are more or less the same as long as the pseudo-ranges have similar paths
from the GNSS antenna site to the satellite. As an example, pseudo-ranges from
two sites separated by less than 50 km to the same satellite will probably have
similar atmospheric conditions and therefore similar errors. The paths through
the troposphere and the ionosphere are more or less the same with respect to the
satellite orbit and geometry. This holds true since the distances to the
satellites are more than 20,000 km compared to a 50 km separation or less for
two stations on the ground.
The first step in differential GNSS is the generation of
single-differences (Figure 4). In this example, a pseudo-range from site one
P1 to satellite one S1
is subtracted from the pseudo-range from site two P2
the same satellite S1. Using this
technique the error sources associated with the satellite orbit, satellite
clock, ionosphere and troposphere are reduced or even eliminated if the same
identically oriented antennas are used on both sites. Although most of the
errors have been reduced, most of the software packages difference a second
time. Here the single-differences are differenced a second time to get a
double-difference (Figure 5). The single-difference to satellite one S1
is subtracted from the single-difference to satellite two S2.
Using this approach, the influence of troposphere and ionosphere are further
reduced and the receiver clock error is eliminated.
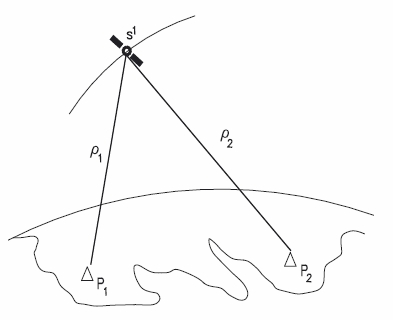
Fig. 4: Single-difference.
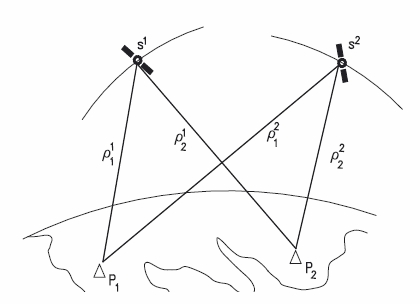
Fig. 5: Double-difference.
If one uses double-differences as measurements in an adjustment
procedure, the relative coordinates between the two sites can be determined. To
get absolute coordinates in the World Geodetic System 1984, (WGS84) – the GPS
coordinate system or the International Terrestrial Reference Frame (ITRF),
coordinates of one of the two sites have to be known. In general the respective
site is called a reference or base station. The second station, for which the
coordinates will be determined, is called the rover. The reference site may be a
single reference site or even a large network of GNSS reference sites (see
Section 4.3). The second approach is significantly more accurate and reliable.
Additionally, it should be mentioned that this technique was originally
developed for post-processing but is now frequently used in real time
positioning.
3.3 Precise Point Positioning
In chapter 3.2 the authors emphasized the importance of relative
GNSS positioning to meet survey specifications. During the last few years a
technique that relies on absolute positioning was developed and continues to
gain in importance – precise point positioning (PPP). The elimination of errors
by differencing is replaced by precisely modeling many of the error sources. The
coordinates of a site are determined with respect to the orbits of the
satellites, similar to absolute positioning, but the orbits are known with a
high level of accuracy (within a few cms). The same is valid for the satellite
clocks (up to sub-nsec). Table 1 gives an overview of the different orbit
classes generated by the International GNSS Service (IGS) (see Appendix A).
Obviously the highest accuracy may be achieved using the IGS final products
where the accuracy of the orbit class improves over time (predicted, rapid,
final). This is one reason why highly accurate results are only possible in
post-processing modes. Results in real time or near real time are published, but
so far the accuracy of the solutions and the reliability of the techniques do
not approach the level needed by many surveying applications.
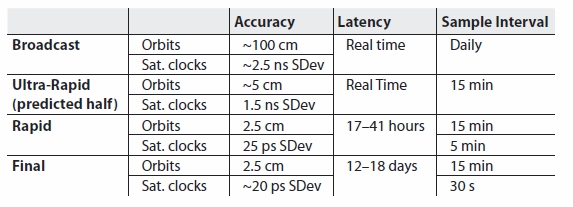
Tab. 1: Orbits and clocks provided by the IGS, broadcast for comparison.
Now returning back to the exact models, three error sources that
require special attention are due to the ionospheric and tropospheric influences
as well as receiver antenna phase patterns. Additionally, there are error
sources that are completely eliminated by using double-differences or absolute
GNSS techniques. Some errors are less importance for PDGNSS because they have no
effect on the accuracy level. Special attention should also be given to phase
centre offsets and variations of the satellite antennas, the phase wind-up at
the satellites, the solid earth tides as well as ocean loading effects.
Table 2 gives an overview on several large error sources and
their influences on the positioning result. These errors can be modeled and thus
the accuracy of PPP using phase measurements is comparable to PDGNSS. The only
disadvantage is that the measurement times need to be longer than in PDGNSS
because each of the different parameters of the models have to be estimated.
Measurement times of 30 minutes are typically required to reach PDGNSS accuracy
levels (convergence time). The main reason for this pertains to the tropospheric
parameters.

Tab. 2: PPP correction models and their impact on positioning.
3.4 Positioning Software Packages and Data
Types
Most manufacturers of resource and geodetic quality GNSS
receivers also provide various software programs to plan survey missions,
collect field and receiver data as well as process the information to produce a
variety of end products such as vectors between reference marks, coordinates and
metadata for GIS and database applications. Larger integrated software systems
used for data collection and processing for survey and engineering projects are
designed to simplify all aspects of a project so data can be adjusted and used
seamlessly by other applications later on. Table 3 lists several common software
packages available to process GNSS data.
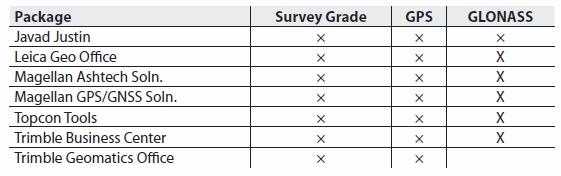
Tab. 3: Exemplary GNSS survey processing packages.
Positioning software packages can typically import two or more
types of GNSS receiver data. A receiver’s native format is usually a proprietary
format that a manufacturer has developed for a number of receiver models from a
production line, such as those used for high-end or geodetic quality
positioning. Software products developed by receiver manufacturers usually
import and process data in the proprietary format. However, if GNSS data from
different brands of receivers are to be processed, then for the most part, a
utility program will need to convert the data to a common format before being
imported.
One of the most common formats for GNSS data processing is RINEX
(Gurtner and Mader, 1990) which is an acronym for Receiver Independent Exchange
format (see Appendices B.13–16). GNSS survey campaigns often have many stations
with numerous receiver and antenna combinations from several manufacturers. One
of the easiest approaches is to work with the data in a common format. The
second benefit to using RINEX data is that it is an ASCII format so a user can
easily view the GNSS observations and other metadata in the RINEX file. A very
good and freely available tool for converting manufacturers’ native receiver
formats to RINEX is TEQC which was developed and is maintained by UNAVCO, a
non-profit membership-governed consortium which facilitates geoscience research
and education using geodesy. The TEQC utility, documentation and a tutorial can
be downloaded from the web site listed in Appendix B.19.
There are also a limited number of high end processing packages
which have been developed by government and academic institutes over that last
decade. These packages offer sophisticated processing techniques and algorithms
and are primarily used to process GNSS data from small and large networks
collected under a variety of conditions. These programs are also used for
satellite orbit determination by several IGS analysis centers on a daily basis.
4. Cost-effective GNSS
4.1 Introduction
Since this report primarily pertains to cost-effective GNSS, the
main question that arises is how to economize ones financial and physical
resources without losing quality. In general a surveyor has to work as
accurately as necessary to meet the requirements given by the principal, not as
accurate as possible. In other words, efficient technologies and techniques
should be used to generate well-qualified products which meet the needs of the
surveyors. Within this report, two possibilities to economize resources will be
described in more detail.
The first possibility (see Section 4.3) is the use of
Continuously Operating Reference Stations (CORS) or even CORS networks. In this
case, the expenses for the master or reference station can be avoided. This
implies that the costs for the hardware as well as the salaries for staff to
operate the reference station may be saved. Besides, the organizational effort
is reduced significantly since the surveyor may act as though he performs an
absolute GNSS survey. In most countries, the surveyor usually pays for PDGNSS
services provided by CORS network service provider or authority. Nevertheless
the benefit provided is often enormous.
The second possibility (see Section 4.2) is to use GNSS receivers
and antennas that are less expensive than “standard” geodetic equipment. The
utilized receivers may be navigation or resource grade receivers or even
OEM-boards or small GNSS or GPS modules. These types of receivers have low
prices which start at around 150 €. Users may economize the price difference to
a geodetic receiver, if he knows how to handle the respective hardware and
software. These types of receivers may be used in combination with a CORS
network in real time as a rover receiver to combine the two price advantages.
4.2 Cost-Effective Rovers / Low-Cost GNSS
Receivers
Normal geodetic GNSS surveys are based on high-quality GNSS
receivers and antennas. Frequently, the surveying community uses dual-frequency
receivers to solve the ambiguities faster and more reliably. In the last few
years, single-frequency receivers have proved to work very reliably if baseline
lengths are below 10 km to 15 km. This opens up the market for receivers that
are used for navigation since these receivers generally have a single frequency.
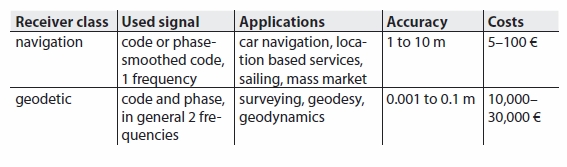
Tab. 4: Characteristics of geodetic and navigation GNSS.
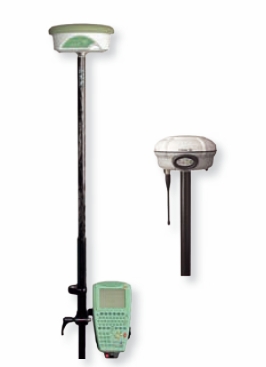
Fig. 6: Exemplary geodetic receivers.
Table 4 compares the characteristics between navigation and
geodetic quality receivers. In general navigation type receivers do not use the
phase data. This problem is overcome by some manufactures where they provide
access to the code and phase measurements from the raw via a serial or a USB
interface. Some of manufacturers (e.g. u-blox) are officially documenting their
format while others (e.g. Garmin) do not provide official format information or
guarantee that the format will exist in the future. Finally, some manufacturers
(e.g. Sirf ) document their phase data format but do not provide access to the
data for the users. With respect to geodetic quality receivers, a real time
solution cannot be provided. Currently the raw carrier phase and code data are
transformed into RINEX data and stored before it can be used efficiently. For
the u-blox Lea-4T, the conversion from raw to usable observables may be carried
out using the software teqc (see Appendix B.19).
For geodetic applications, highly precise antennas such as the
micro-strip and choke rings are commonly used. They are constructed to reduce
multipath effects and phase centre variations as well as type specific
variations regarding the antenna phase centre offset. These choke ring antennas
may cost up to 10,000 €. Sometimes the GNSS receiver and antenna are integrated
as one unit. In contrast, many navigation type receivers integrate low-priced,
simple antennas directly into their receiver box, while some receivers simply
connect to an external antenna via a cable. In the latter case, the antenna may
be fixed such as on the roof of a car using a magnet on the antenna casing.
Portable antennas usually range in price but start at several €s or $s. In
general however, an antenna and a receiver are sold as a package.
The quality of the performance of navigation type receivers can
be improved if precise geodetic antennas are used. In this example, the
cost-effectiveness is clearly reduced, so in this report the combination of
navigation type receiver and navigation type antenna is mainly considered. The
advantage of precise geodetic antennas can be reduced or even eliminated if the
navigation type antennas are calibrated. Figure 11 shows an example for the
u-blox ANN-MS antenna (in combination with the u-blox LEA 4T receiver).
Additionally, the navigation antennas need the capability of being leveled and
centered. Figure 10 shows an adapter that combines geodetic style equipment,
such as the Leica tribrack, with a u-blox ANN-MS antenna. Some experiments show
that metal ground plates often reduce multipath effects, especially when metal
reflectors such as a car roof are nearby.

Fig. 7: Garmin eTrex Vista (raw phase data available, format not
documented). |

Fig. 8: u-blox Lea-4T receiver (raw phase data available, format
documented). |
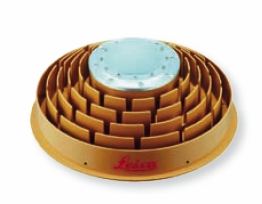
Fig. 9: High precision choke ring antenna (source: Leica
Geosystems).
|
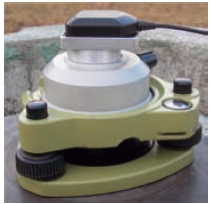
Fig. 10: Low-price u-blox ANN-MS antenna
with adapter. |
In the following section, an overview on low-cost,
receiver-antenna combinations are given. The entries in the table are given as
an example, mainly because the content and number of combinations in the table
will change rapidly in the future. The overview is restricted to exemplary
Garmin and u-blox receivers for prices up to 150 € for a receiver-antenna
combination. Garmin offers more receiver types which provide phase data in a
documented or non-documented way.

Fig. 11: Antenna pattern for u-blox ANN-MS antenna.

Tab. 5: Overview on low-cost GNSS receivers with available phase data.
The accuracy of coordinates determined by low-cost receivers is
very similar to those obtained from geodetic type receivers. Hill et al. (2001)
reports standard deviations below the dm level for Garmin receivers. Schwieger
documents accuracies around 2 cm for baselines up to 1.1 km using a Garmin eTrex
receiver (Schwieger, 2007 and Schwieger & Wanninger, 2006) and below 2 cm for
baselines up to 7 km using a u-blox AEK-4T receiver (Schwieger, 2009) with
observation times between 20 and 30 minutes.
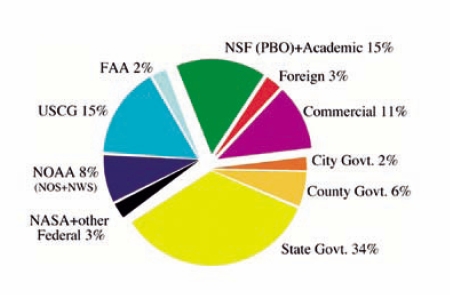
Fig 12: Federal, state, local, commercial and academic participants of
the CORS network in the United States.

Fig 13: Sectors who use GNSS data on a routine basis.
ABIDIN & MUCHLAS (2005) obtain standard deviations below 20 cm
for baselines up to 100 km in length with 20 minutes occupation time. In
conclusion, the accuracy is not equivalent to single- or dual-frequency geodetic
receivers but for many surveying applications the level is sufficient.
4.3 Continuously Operating Reference Station
(CORS) Networks
4.3.1 Introduction
In 1994, William E. Strange, the Chief Geodesist of the National
Geodetic Survey, was the first individual who defined the term Continuously
Operating Reference Station (CORS) as a permanently installed geodetic quality
receiver and antenna positioned over a monument or point which collected GPS
data 24 hours a day, every day of the year. The initial idea was to establish a
network of CORS so users could use data from any of the permanent stations with
their own GPS equipment. CORS networks typically have GNSS receivers which
provide carrier phase and code range measurements in support of 3-dimensional
positioning activities. Today there are numerous CORS networks which have been
established throughout the world (see Appendix C) to support an unlimited number
of applications.
Engineers, surveyors, GIS/LIS professionals, scientists and
others can apply CORS data to position points at which GNSS data have been
collected as well as using the data to model a number of physical systems. A
CORS system enables positioning accuracies that approach a centimeter or better
relative to a worldwide network, such as the ITRF or to a local network such as
the NAD83 in the United States. CORS systems benefit from a multi-purpose
cooperative endeavor involving numerous governmental, academic, commercial and
private organizations. As an example, the diagrams shown below illustrate the
agencies and sectors that participate in the National CORS network of the United
States.
4.3.2 CORS Station Configuration
The size and complexity of a CORS network varies considerably and
therefore one station design cannot address all configurations. However, the
following areas should be considered when planning new CORS or expanding an
existing network. GNSS receivers should be able to track multiple constellations
such as simultaneously collecting data from the GPS and GLONASS systems. The
receiver should also use a geodetic quality antenna, preferably one that
minimizes multipath and is mounted to a pillar or other stable structure. For
remote installations, an enclosure to house power supplies, batteries, a
computer and telecommunications equipment is strongly suggested. If the CORS
station is part of a mission-critical program such as the Wide Area Augmentation
System (WAAS) offered by the Federal Aviation Administration (FAA), then
installing multiple receivers at a location is worth considering. The following
figure below depicts a typical CORS installation from the Plate Boundary
Observatory (PBO) in the Western United States.
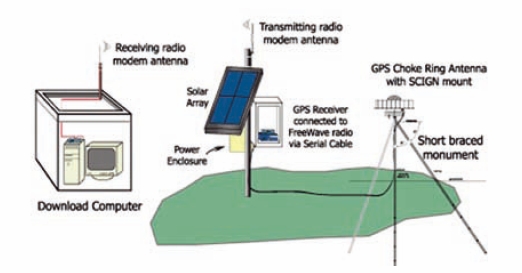
Fig 14: Typical CORS station configuration for the Plate Boundary
Observatory (PBO) network. Courtesy UNAVCO, CO.
4.3.3 Products from a CORS Network
For large CORS networks, the design may include regional data
centers for quality checking, processing, distributing and archiving GNSS data.
Most networks around the world collect and disseminate GNSS data 24 hours a day
as well as offering other services and IGS products such as broadcast and
precise ephemerides and clock information for post processing (see Appendix A).
There are also an increasing number of data centers which offer
real time RTCM-104 format data streams using the Networked Transport of RTCM via
Internet Protocol (Ntrip) (see Appendix B.6, B.10). Ntrip is an open protocol
based on the Hypertext Transfer Protocol HTTP/1.1 and has many advantages such
as having the ability to stream any kind of GNSS data, disseminating numerous
streams simultaneously and can be used over mobile IP networks using TCP/IP.
Data centers which offer RTCM or real time data streams usually have a server
known as an NtripCaster that listens for requests from users NtripClients
for one or more data streams. The data streams are then used to support
stationary or mobile applications such as rapid static and kinematic surveys,
hydrography, LIS/GIS development and vehicle navigation. These types of data
centers will play a more significant role as the need for faster and more
readily available GNSS information is desired.
4.4 Web-based Positioning Tools
A rapid and automated use of CORS networks are implemented in
many post-processing services. In this case, a user does not need to worry about
the processing tasks involved. A user sends their data, usually in RINEX format,
to the service provider, a solution is computed and the estimated coordinates
are sent to the user via email. The following sections will give an overview of
four different services that are currently available for use.
4.4.1 Online Positioning User Service – OPUS
The Online Positioning User Service (OPUS) from the National
Geodetic Survey is a web-based service to provide GPS users with an easy method
to submit and process their data in an accurate and reliable fashion. The end
products are two sets of geodetic coordinates having a precision of about 1.0 cm
and are consistent with the latest ITRF coordinate system and the National
Spatial Reference System (NSRS) of the United States.
To use OPUS, a user needs to provide the name of the raw or RINEX
data file, select an antenna type from a pull-down menu, enter the antenna
height and provide an email address to which the report will be mailed. Once the
data has been upload and verified, a web page reports the data has been
submitted successfully and a user should expect his or her results via email a
few minutes later. OPUS then uses L1 and L2 carrier phase data from the rover
and the best three CORS stations in the vicinity of the rover for processing.
The CORS stations are usually from the National CORS network if a rover dataset
was collected in the United States or from the IGS network if the rover was
collected in a foreign country or region.
OPUS relieves users of the burden of processing their own data by
providing a simple interface and rapid turnaround. The service processes about
20,000 datasets a month and has over 60,000 unique users. OPUS has been used to
support numerous applications with a few of the most popular being to support
construction and engineering projects, surveying, mapping, mining and
spaced-based imagery.
To learn more about OPUS or begin using the services offered by
the National Geodetic Survey, please visit the web page listed in Appendix B.23.
4.4.2 Scripps Coordinate Update Tool – SCOUT
The Scripps Coordinate Update Tool (SCOUT) is also a web-based
geodetic tool that can be used to compute a set of coordinates for a station.
SCOUT assumes the data is submitted in RINEX format which could be normal or
Hatanaka-compressed observation files that may be further compressed using the
traditional UNIX compress, gzip or bzip utilities.
SCOUT uses the GAMIT processing engine from the Department of
Earth Atmospheric and Planetary Sciences, MIT to process the submitted dataset
with CORS data from the three closest stations. A least squares network
adjustment is performed with the rover and CORS and upon completion,
coordinates, statistics and a regional map which shows the location of the
stations are emailed to the user. The Cartesian coordinates are referenced to
the ITRF05 reference frame while the geodetic coordinates are referenced onto
the World Geodetic System 1984 (WGS84). For additional information on the SCOUT
program offered by the Scripps Orbit and Permanent Array Center, Scripps
Institute of Oceanography, please see the web link at Appendix B.24.
4.4.3 AUSLIG’s Online GPS Processing Service
– AUSPOS
AUSPOS is also a web-based positioning utility which provides
users the ability to submit GPS data to a processing system. This free service
accepts static, dual frequency, geodetic quality data and makes use of the
Geocentric Datum of Australia (GDA) and the International Terrestrial Reference
Frame (ITRF). AUSPOS also uses a number of IGS products in the processing phase
to produce an accurate and consistent set of coordinates for data collected
anywhere on the globe.
AUSPOS has been specifically tailored to simplify several
surveying and engineering tasks such as positioning DGPS and remote GPS
reference stations, determining ultralong GPS baselines, establishing geodetic
connections to IGS and ARGN stations and for performing GPS network quality
control. To use the service, a user typically submits the antenna type and
height, an email address and RINEX data, up to 24 hours in duration, to a
website for processing. A set of the closest IGS reference stations are then
used in a double difference approach to estimate the best set of coordinates for
the remote dataset while holding the remaining IGS stations fixed. The results
are provided in an email as well as through a link to the AUSPOS anonymous ftp
server which stores the results. For additional information on AUSPOS, please
visit the web address referenced in Appendix B.25
4.4.4 Canadian Spatial Reference System
Online Global GPS Processing Service – CSRS-PPP
Natural Resources Canada (NRCan) provides a precise point
positioning (PPP) service through the web which can compute highly accurate
positions from raw GPS observation data in a post-processing mode. The PPP
system uses precise IGS orbit and clock information and can accept static or
kinematic data from either single or dual frequency receivers. The processing
algorithm determines what observables are available and then proceeds with one
of two scenarios. The first approach is to use L1 and L2 pseudorange and carrier
phase data to obtain a solution. If the first approach fails or if the data only
contains a single frequency, then an L1 pseudo-range solution will be performed.
The PPP processing system was designed to simplify GPS processing
by providing a minimum number of requirements to address. A user needs to submit
a RINEX file, select the type of processing desired and the reference frame the
coordinates are to be reported in. Currently the PPP service will produce
coordinates referenced either to the NAD83 (CSRS) or to the ITRF systems. A
successful solution will produce an email sent to the user which contains a link
to two forms of output. The summary reports can be short or extended and contain
statistical information as well as the coordinates. A time series plot
containing the estimated parameters and corresponding standard deviations is
also available for review and downloading. For more information on NRCan’s PPP
system, please refer to the web link in Appendix B.26.
5. Cost-effectiveness
After a description of the technical details given in the
proceeding chapters, this chapter highlights the economic benefits associated
with the reduction of the working costs by implementing the techniques described
before. The estimation of the financial benefit cannot be 100% correct since the
labor costs are quite different in most countries. For this reason, approximated
values and intervals are introduced and shown in the following figures. We use
an interval from 1 € (lowest level, developing countries) to 70 € (developed
countries) to get a rough estimation.
As a first example, the benefit of a using a CORS reference
station network is presented. For this variant, the surveyor economizes the
financial resources to be spent for the receiver at the reference site and for
one person to assemble and care for the reference receiver during the
measurement stage of the survey. Geodetic dual-frequency receivers having a
price of 20,000 € are used for the comparison. It is assumed that a receiver can
be used for three years and would therefore give a 6,666 € per year operational
cost. Two variants could be investigated. The first is when the service is free
of charge and the second is when you have to pay for it. For the second case an
interval from 500 € up to 3,000 € per year as possible flat rates may be
considered. Figures 16, 18 and 19 reflect a flat rate of 1,000 € per year.
Figure 15 shows the costs per year for the case where no cost-effective
techniques are used. Figure 16 presents the case where the costs of integrating
the receiver into a CORS network are considered. For both figures, the costs are
estimated for different labor costs which range from 1 € and 70 € per hour.
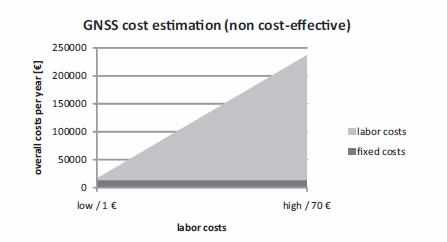
Fig 15: Costs per year for geodetic positioning using GNSS standard
configuration.
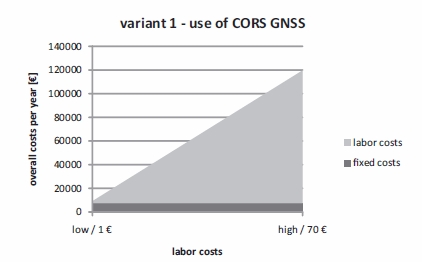
Fig 16: Costs per year for geodetic positioning applying CORS
integration.
The second benefit is achieved when low-cost receivers are used
for data acquisition. In this case, the amount for the rover and the reference
receiver is reduced significantly, usually between 20,000 € to approximately 100
€. The labor costs do not change, but there may be additional costs such as for
two laptop computers or data loggers (overall approximately 2,000 € in 3 years)
have to be considered. Software is an additional expense and is available for
1,000 €. Figure 17 presents the costs for this variant.
The third possibility is the combination of both, the use of
low-cost receivers with a CORS network. For this variant shown in figure 18, the
assumptions given above are still valid.
Obviously Figures 15 through 18 make it difficult to visualise
what effect different labor costs have. Figure 19 therefore illustrates the
benefits of the different variants (Figures 16–18) with respect to the GNSS
standard configuration (Figure 15) for three labor cost levels (1 €, 10 € and 70
€). The benefits and cost savings in using a CORS station or network is
important in many cases, but for developed countries it is most beneficial
because labor costs are high and these are the ones to be optimized in this
variant. Variant 2 is most important for very low labor costs but becomes less
attractive with increasing labor costs, especially when they are given as a
percentage. The benefit is further improved for the combination of both
variants.

Fig 17: Costs per year for geodetic positioning using Low-Cost GNSS.
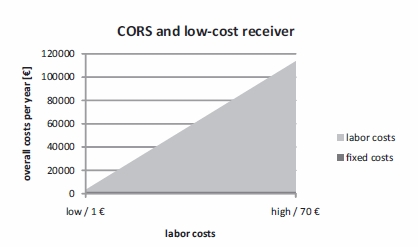
Fig 18: Costs per year for geodetic positioning using CORS and Low-Cost
GNSS.

Fig. 19: Benefit of the different cost-effective techniques.
6. Concluding Remarks
FIG Commission 5 – Positioning and Measurement would like to
emphasize that there are many possibilities when performing static and kinematic
surveys and are encouraged by the results delivered by current GNSS positioning
technologies. The reliability, promptness and accuracy of the hardware and
techniques will continue to increase in the future, especially when the number
of available satellites continues to grow from year to year.
The main focus of the report was to describe the use of this
emerging technology in a cost-effective way and to illustrate the cost
advantages of these technologies. The advantages shown will hopefully encourage
surveyors all over the world to establish cost-effective surveying practices
using GNSS positioning within their profession.
Appendix A – The International GNSS
Service
History
The International GNSS Service (IGS) is a voluntary scientific
organization which was officially founded in January 1994. The IGS, originally
known as the International GPS Service, was renamed in 2005 because other
operational (GLONASS) and planned (Galileo) Global Navigation Satellite Systems
(GNSS) were increasingly playing a more significant role. Today the IGS is a
service of the International Association of Geodesy (IAG) and consists of more
than 200 participating organizations from approximately 80 countries whose
primary mission is to collect, archive and disseminate high-quality GPS and
GLONASS data and associated products (Beutler et al. 1999, 2009, Dow et al.
2009).
Many of the products offered through the IGS are derived from
satellite observation data taken directly from the global IGS tracking network.
As of May 2009, approximately 300 continuously operating reference stations
(CORS) form the network, with many of the stations providing data in real-time
or near real-time to a central processing center. The data are then used to
compute precise and highly accurate geodetic measurements to support numerous
scientific fields such as geodesy, geodynamics, engineering, oceanic and
atmospheric research, navigation, and surveying and mapping. The first few
products to become freely available from the IGS were the GPS satellite
ephemerides, GPS clock corrections and the earth rotation parameters (ERP).
GLONASS products started to show up during 1998 after a sufficient number of
satellites were in orbit. Additional products such as troposphere and ionosphere
data, reference station data in RINEX format (Gurtner et al. 1990, 2002),
reference station coordinates and velocities were added later and are now freely
available through the IGS Central Bureau (see
http://www.igs.org/components/prods.html) and several global and regional
analysis centers. Although the majority of the products produced are used by
academic and research institutes for scientific investigations, several products
such as satellite ephemerides and RINEX data are heavily used by the engineering
and surveying communities. These types of data are also compatible with numerous
third party GIS, engineering and surveying software products and are used in
many different applications.
Today numerous users have adopted many of the IGS products for
use in cm-level geodetic positioning applications as well as using the products
for maintaining a highly accurate International Terrestrial Reference Frame
(Altamimi et al. 2009). Users will continue to benefit from the improvements and
timeliness of these products as more accurate positioning and navigation
applications become part of the mainstream.
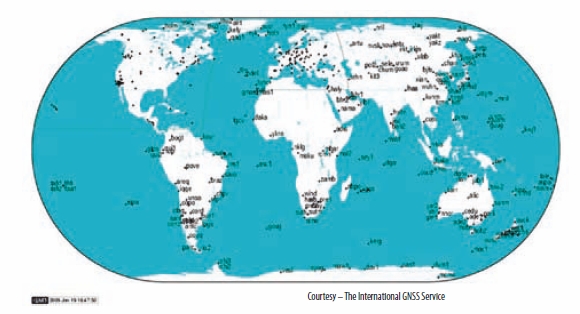
Ongoing Projects
Clock Products
Currently there are seven IGS analysis centers which produce
clock solutions on a daily basis. Theses clock solutions are used by the IGS
Clock Product Coordinator to form two IGS timescales, the new Rapid IGS
timescale (IGRT) and the new Final IGS timescale (IGST). The IGS Rapid and Final
products are then aligned to these timescales. For additional information on the
IGS clock products, see
https://timescales.nrl.navy.mil/IGStime/index.php, (Ray et al. 2003).
Real Time Pilot Project
The concept of a real time network within the IGS infrastructure
originated at a workshop titled Towards Real-Time in Ottawa, Canada in 2002.
Since that time a prototype network referred to as the Real-time Pilot Project
was developed and became operational with over 50 active stations. As of April
2009, there are two operational systems to deliver real time data to the users.
The first system, UDPRelay, is operated by Natural Resources Canada (NRCan) and
provides data streams from 50 stations using the UDP protocol. The second system
uses the Bundesamt für Kartographie und Geodäsie (BKG) NTRIP infrastructure and
transmits data from reference stations to several broadcast servers known as
NtripCasters. Data from 150 reference stations can now be accessed via the HTTP
protocol from the IGS Caster at www.igs-ip.net.
Please refer to the following web addresses for additional information on the
two real-time networks.
http://www.rtigs.net/architecture.php
http://igs.bkg.bund.de/index_ntrip.htm
Reference Frame Working Group
The Reference Frame Working Group is primarily tasked with
generating the coordinates and velocities for the reference stations in the IGS
global network, computing daily EarthRotation Parameters (ERP) and weekly
estimates for the geocenter, as well as producing the corresponding covariance
information. The computations are usually aligned to the International
Terrestrial Reference Frame (ITRF) and performed on a regular basis. Daily
solutions are routinely „stacked“ in a cumulative fashion to obtain a more
accurate set of coordinates and velocities at a specific epoch. The Reference
Frame Working Group also strives to design efficient algorithms and accurate
models to produce the best set of products for the IGS. For additional
information on IGS station products, please visit the following web page.
http://igscb.jpl.nasa.gov/projects/reference_frame/index.html
Ionosphere Working Group
The IGS Ionosphere Associate Analysis Centers (IAAC) use varying
techniques to produce independent rapid and final Vertical Total Electron
Content (TEC) maps and datasets as well as producing combined versions of both
products. Regional and global TEC maps are widely used by many scientific fields
and commercial communications industries for monitoring the ionosphere,
calibrating positioning and navigation systems and for applying corrections due
to signal delays experienced from satellites above the ionosphere. The figure
below was created in real time with data received from 100 global tracking sites
and shows a dynamic ionosphere on August 26, 2009; Courtesy Jet Propulsion
Laboratory, California Institute of Technology.
Troposphere Working Group
There are currently nine Associate Analysis Centers which are
involved in producing atmospheric products related to the troposphere and the
ionosphere. These two regions of the atmosphere are fairly dynamic and each can
have a significant impact on GNSS signals. The main product of the Troposphere
Working Group is an estimate for the total zenith path delay computed from the
reference stations in the IGS global network. If additional measurements such as
temperature and pressure are taken at ground level near a reference station,
then it is possible to estimate the precipitable water vapor from the zenith
path delays. The precipitable water vapor is of particular importance to groups
working in meteorology and climatology since the quantity of water vapor in the
atmosphere determines the type of weather forecast given for the next 6 to 12
hours. Longer term monitoring of water vapor from a regional point of view is
also important for estimating climate change. For additional information on this
topic, please visit the following web page.
http://igscb.jpl.nasa.gov/projects/tropo/index.html
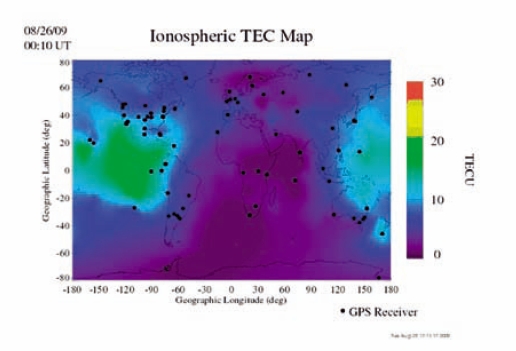
Products

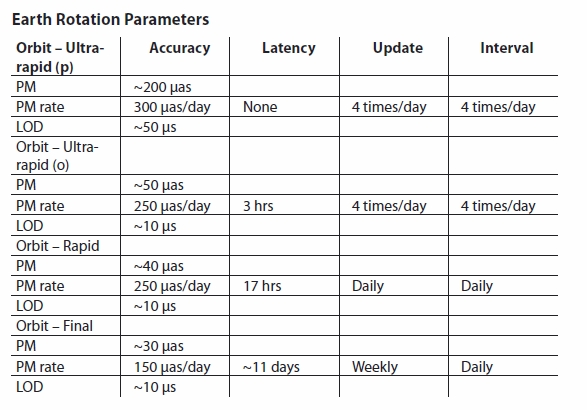
IGS Tracking Network
http://igscb.jpl.nasa.gov/network/netindex.html
Appendix B – Additional Information and
Resources
-
The International GNSS Service (IGS).
http://www.igs.org
-
IGS Central Bureau (IGSCB)
http://igscb.jpl.nasa.gov/
Crustal Dynamics Data Information System (CDDIS)
http://cddis.nasa.gov/ftpgpsstruct.html
International Assocaition of Geodesy
http://www.iag-aig.org
-
The International Earth Rotation and Reference Systems
Service
http://www.iers.org
-
Networked Transport of RTCM via Internet Protocol (NTRIP)
http://igs.bkg.bund.de/index_ntrip.htm
-
IGS Real Time Working Group
http://www.rtigs.net/architecture.php
-
IGS Clock Products Working Group
https://goby.nrl.navy.mil/IGStime/
-
Natural Resources Canada, Earth Sciences Sector
http://ess.nrcan.gc.ca/geocan/centres_e.php
-
The Radio Technical Commission for Maritime Services
http://www.rtcm.org/
-
Earth Rotation Parameters Format
http://igscb.jpl.nasa.gov/igscb/data/format/erp.txt
-
IONEX: The IONosphere Map Exchange Format
http://igscb.jpl.nasa.gov/igscb/data/format/ionex1.pdf
-
RINEX 2: Receiver Independent Exchange Format
http://igscb.jpl.nasa.gov/igscb/data/format/rinex2.txt
-
RINEX 2.10
http://igscb.jpl.nasa.gov/igscb/data/format/rinex210.txt
-
RINEX 2.11
http://igscb.jpl.nasa.gov/igscb/data/format/rinex211.txt
-
RINEX 3.00
http://igscb.jpl.nasa.gov/igscb/data/format/rinex300.pdf
-
SINEX: Solution (Software/technique) Independent Exchange Format
http://www.iers.org/MainDisp.csl?pid=190-1100110
-
NAVSTAR Global Positioning System Interface Specification
IS-GPS-200D
http://www.navcen.uscg.gov/gps/geninfo/IS-GPS-200D.pdf
-
TEQC – The Toolkit for GPS/GLONASS/Galileo/SBAS Data
http://facility.unavco.org/software/teqc/teqc.html
-
UNAVCO
http://www.unavco.org/
-
European Space Agency – Galileo
http://www.esa.int/esaNA/galileo.html
-
Russian Space Agency – GLONASS
http://www.glonass-ianc.rsa.ru/pls/htmldb/f?p=202:1:9939630416051479874
-
Online Positioning User Service – OPUS
http://www.ngs.noaa.gov/OPUS/
-
Scripps Coordinate Update Tool – SCOUT
http://sopac.ucsd.edu/cgi-bin/SCOUT.cgi
-
Online GPS Processing Service – AUSPOS
http://www.ga.gov.au/geodesy/sgc/wwwgps/
-
Canadian Spatial Reference System – PPP
http://www.geod.nrcan.gc.ca/products-produits/ppp_e.php
Appendix C – Global and Regional
Reference Station Net Works
Global
-
IGS Tracking Network
http://igscb.jpl.nasa.gov/network/netindex.html
North America
-
The National CORS Network – United States
www.ngs.noaa.gov/CORS/
-
Plate Boundary Observatory – Western United States
http://pboweb.unavco.org/
-
The Southern California Integrated GPS Network
http://www.scign.org/
-
The Western Canada Deformation Array
http://gsc.nrcan.gc.ca/geodyn/wcda/index_e.php
-
The Canadian Spatial Reference System
http://www.geod.nrcan.gc.ca/cacsname_e.php
-
Bay Area Deformation Array – USGS/UC Berkeley
http://www.ncedc.org/bard/
-
Eastern Basin-Range and Yellowstone Hotspot GPS Network
http://www.earth.utah.edu/people/faculty/rsmith
-
Pacific Northwest Geodetic Array
http://www.panga.cwu.edu/
-
Parkfield, California Crustal Deformation Measurements
http://earthquake.usgs.gov/research/deformation/monitoring/
Central America
-
Red Geodésica Nacional Activa – Mexico
http://www.inegi.org.mx/inegi/default.aspx?s=geo
South America
-
Estaciones GNSS Permanentes – Argentina
http://www.copa.org.ar/Eljalon/estaciones.htm
Europe
-
SAPOS ® – German National Survey Satellite Service
Positioning in Berlin
www.stadtentwicklung.berlin.de/geoinformation/sapos/
-
SWEPOS – Swedish Network of Permanent Reference Stations for GNSS
http://swepos.lmv.lm.se/
-
EUREF Permanent Network – Europe
http://www.epncb.oma.be/_trackingnetwork/
-
Geodetic Data Archiving Facility – Italy
http://geodaf.mt.asi.it/html_old/index.html
-
Réseau GPS Permanent – France
http://rgp.ign.fr/
-
Automated GNSS Network for Switzerland
http://www.swisstopo.admin.ch/internet/swisstopo/en/home/topics/survey/permnet/
agnes.html
-
CZEPOS – Czech Republic
http://czepos.cuzk.cz/
-
ESTPOS – Estonia
http://www.maaamet.ee
-
GPSNET.HU – Hungary
http://www.gpsnet.hu
-
LATPOS – Latvia
http://www.latpos.lgia.gov.lv
-
LITPOS – Lithuania
http://eupos.vgtu.lt/
-
ASG-EUPOS – Poland
http://www.asg-eupos.gov.pl
-
ROMPOS – Romania
http://www.rompos.ro
-
AGROS – Serbia
http://www.agros.rgz.gov.rs
-
SKPOS – Slovak Republic
http://www.skpos.gku.sk
-
SIGNAL – Slovenia
http://www.gu-signal.si
Africa
Asia
-
Geographical Survey Institute – Japan
http://www.gsi.go.jp/ENGLISH/page_e30030.html
Australia and New Zealand
-
Australian Regional GPS Network
http://www.ga.gov.au/geodesy/argn/
-
GeoNet – New Zealand
http://www.geonet.org.nz/index.html
-
PositioNZ – New Zealand
http://www.linz.govt.nz/geodetic/positionz/index.aspx
- Abidin, H.Z., Muchlas, A. (2005): GPS Surveying using Navigation Type
Receivers. South East Asia Survey Congress 2005, 21–25 November, Bandar Seri
Begawan, Brunei.
- Altamimi, Z., Collilieux, X., Legrand, J., Garayt, B. and Boucher, C.
(2007) ITRF2005: A new release of the International Terrestrial Reference
Frame based on time series of station positions and Earth Orientation
Parameters. J. Geophysical Research 112:1–19
- Beutler, G., (2005) Methods of Celestial Mechanics. Volume I: Physical,
Mathematical, and Numerical Principles. Springer Berlin, Heidelberg, New
York.
- Beutler, G., Moore, A.W. and Mueller, I.I. (2009) The international
global navigation satellite systems service (IGS): development and
achievements. J. Geodesy 83:297–307.
- Beutler, G., Rothacher, M., Schaer, S., Springer, T., Kouba, J., and
Neilan, R.E. (1999) The International GPS Service (IGS): an
interdisciplinary service in support of earth sciences. Adv. Space Res.
23:631–653.
- Dow, J.M., Neilan, R.E., and Rizos, C. (2009) The International GNSS
Service in a changing landscape of Global Navigation Satellite Systems. J.
Geodesy 83:191–198.
- Gibbons, G. (2009) GLONASS Builds Monitoring, Augmentation Systems.
Inside GNSS September/October 12–13.
- Griffiths, J. and Ray, J. (2009) On the precision and accuracy of IGS
orbits. J. Geodesy 83:277–287.
- Gurtner, W. and Mader, G. (1990) Receiver Independent Exchange Format
Version 2. CSTG GPS Bulletin 3, National Geodetic Survey, Rockville MD, USA.
- Gurtner, W. (2002) RINEX: The Receiver Independent Exchange Format
Version 2.10.
ftp://igscb.jpl.nasa.gov/igscb/data/format/rinex210.txt
- Hill, C.J., Moore, T., Dumville, M. (2001): Carrier Phase Surveying with
Garmin Handheld GPS Receivers. Survey Review, pp 135–141.
- Hofmann-Wellenhof, B., Lichtenegger, H. And Collins, J., (1994) GPS
Theory and Practice. Third Revised Edition, Springer-Verlag Wien, New York,
NY, USA.
- Kouba, J. and Héroux, P. (2001) Precise Point Positioning Using IGS
Orbit and Clock Products. GPS Solutions 5:12–28.
- Kouba, J. (2009) A Guide to Using International GNSS Service (IGS)
Products.
-
http://igs.org/igscb/resource/pubs/UsingIGSProductsVer21.pdf.
- Leick, A., (2004) GPS Satellite Surveying. John Wiley & Sons, Inc.,
Hoboken NJ, USA.
- Mader, G.L. and Weston, N.D. (2006) NOAA’s Online Positioning User
Service (OPUS). GeoIntelligence JAN/FEB.
- Noll, C., Bock, Y., Habrich, H. and Moore, A. (2009) Development of data
infrastructure to support scientific analysis for the International GNSS
Service. J. Geodesy 83:309–325.
- Parkinson, B.W., and Spilker Jr., J.J., (1996) Global Positioning
System: Theory and Applications Volume I. American Institute of Aeronautics
and Astronautics, Inc., Washington DC, USA
- Ray, J. and Senior, K. (2003) IGS/BIPM Pilot Project. GPS carrier phase
for time/ frequency transfer and time scale formation, 4th International
Time Scale Algorithms Symposium. 40 (3):S270–S288.
- Rizos, C. (2008) Multi-Constellation GNSS/RNSS from the Perspective of
High Accuracy Users in Australia. Spatial Science 53:29–63.
- Schwieger, V. (2009): Accurate High-Sensitivity GPS for Short Baselines.
FIG Working Week, Eilat, Israel, March 3–8, 2009.
- Schwieger, V. (2007): High-Sensitivity GPS – the Low Cost Future of
GNSS. FIG Working Week, Hong Kong, SAR, 13.–17.05.2007.
- Schwieger, V., Waninger, L. (2006): Potential von GPS
Navigationsempfängern. In: GPS und Galileo. Beiträge zum 66. DVW-Seminar am
21. und 22. Februar 2006 in Darmstadt, Wißner Verlag, Augsburg, 2006.
- SPS. (2001) Global Positioning System Standard Positioning Service
Performance Standard. OASD/C31/ODASD (C31SR&S)/Space Systems. Pentagon,
Washington D.C.
- Stone, W. (2006) The evolution of the National Geodetic Survey’s
Continuously Operating Reference Station network and Online Positioning User
Service, Proc. ION-IEEE Position, Location, and Navigation Symp.
Apr:653–663.
- Strang, G. and Borre, K., (1997) Linear Algebra, Geodesy, and GPS.
Wellesley-Cambridge Press, Wellesley MA, USA.
Published in English
Copenhagen, Denmark
ISBN 978-87-90907-79-2
Published by
The International Federation of Surveyors (FIG)
Kalvebod Brygge 31–33, DK-1780 Copenhagen V
DENMARK
Tel. +45 38 86 10 81
Fax +45 38 86 02 52
E-mail: [email protected]
www.fig.net
January 2010
ACKNOWLEDGEMENTS
Editors: Dr. Neil D. Weston, United States and Dr. Volker Schwieger, Germany
Cover photos, front: GPS IIR satellite, u-blox antenna on pillar (©IAGB)
Design and layout: International Federation of Surveyors, FIG
Printer: Oriveden Kirjapaino, Finland
|